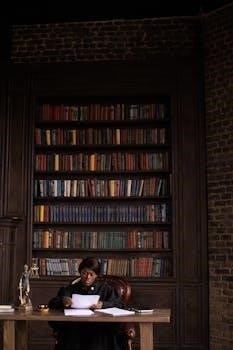
Thermodynamics, a branch of physics, explores the relationships between heat, work, and energy, particularly focusing on macroscopic systems․ It provides fundamental laws that govern how energy is transferred and transformed․ These laws are essential for understanding various phenomena, from engines to chemical reactions․
What is Thermodynamics?
Thermodynamics is a branch of physics that delves into the study of energy and its transformations, specifically focusing on how heat and work relate to each other and to other forms of energy․ It examines the behavior of macroscopic systems, dealing with bulk properties of matter rather than microscopic details․ This field is concerned with understanding how energy moves within systems and between them, encompassing concepts like temperature, pressure, and volume․ Thermodynamics establishes fundamental laws that govern these interactions, offering a framework for analyzing energy conversion, efficiency, and the direction of natural processes․ It’s crucial for various engineering and scientific applications, from designing engines to understanding chemical reactions and material properties․ Thermodynamics is an indispensable tool for comprehending the energy landscape that shapes our world, focusing on the relationship between energy, heat and work at a macroscopic level․
Classical vs․ Statistical Thermodynamics
Thermodynamics can be approached from two distinct perspectives⁚ classical and statistical․ Classical thermodynamics focuses on the macroscopic properties of systems, such as temperature, pressure, and volume, without delving into the microscopic behavior of individual atoms or molecules․ It is primarily concerned with the relationships between these bulk properties and the transfer of energy․ In contrast, statistical thermodynamics seeks to explain these macroscopic properties by examining the behavior of the constituent atoms and molecules․ It uses statistical methods to analyze the collective behavior of a large number of particles, relating microscopic properties to the observed macroscopic behavior․ While classical thermodynamics relies on empirical observations and phenomenological laws, statistical thermodynamics provides a deeper understanding by connecting the macroscopic world to the microscopic world․ Both perspectives offer valuable insights into thermodynamic phenomena, with statistical thermodynamics often providing a more fundamental basis for understanding the classical laws․
The Zeroth Law of Thermodynamics
The Zeroth Law establishes the concept of thermal equilibrium․ It states that if two systems are each in thermal equilibrium with a third system, then they are in thermal equilibrium with each other․ This law is fundamental for defining temperature․
Concept of Thermal Equilibrium
Thermal equilibrium is a state where there is no net flow of heat between two or more systems in contact․ This implies that the temperatures of the systems are equal, and no further macroscopic changes occur․ Imagine placing a hot object in contact with a cold object; heat will flow from the hot object to the cold object until they reach the same temperature․ At this point, they have achieved thermal equilibrium․ The concept is crucial because it allows us to define a consistent temperature scale․ It’s the foundation for understanding how energy is distributed within systems and it enables us to use temperature as an indicator of a system’s state․ This is a prerequisite for understanding other thermodynamic laws․ The concept of thermal equilibrium is fundamental to all branches of thermodynamics․ It also allows us to make predictions about the behavior of systems when they are brought together․ Thermal equilibrium isn’t about the absence of molecular motion, but rather about the balance of kinetic energy․
Temperature as a Property
Temperature is a fundamental property of a system that determines the direction of heat transfer․ It’s a measure of the average kinetic energy of the particles within a substance․ Higher temperatures correspond to greater average kinetic energy, meaning particles are moving more rapidly․ Temperature isn’t the same as heat; heat is the transfer of energy, while temperature is an indicator of the energy state․ It’s an intensive property, meaning it doesn’t depend on the size or amount of the system, unlike extensive properties like volume or mass․ Temperature is critical in establishing equilibrium, and it is defined by the zeroth law of thermodynamics, ensuring its consistency and measurability․ We can use it to predict heat flow between different objects․ This property allows us to use thermometers, which are calibrated using temperature scales, to accurately measure and compare thermal states․ In essence, it’s a macroscopic property linked to the microscopic behavior of matter․
The First Law of Thermodynamics
The first law of thermodynamics is essentially the principle of conservation of energy․ It states that energy can neither be created nor destroyed but can be transformed from one form to another․ This principle is fundamental in understanding energy transfer in systems․
Conservation of Energy
The concept of conservation of energy is a cornerstone of the first law of thermodynamics․ It dictates that within an isolated system, the total amount of energy remains constant․ Energy, in its various forms, such as kinetic, potential, thermal, and chemical, may transform into one another, but the overall sum of all energy types will not change over time․ This principle is invaluable for analyzing processes and predicting outcomes of various physical and chemical interactions․ For instance, when analyzing a system like a heat engine, the total energy input must equal the total energy output, accounting for any work done and heat transfer․ This law provides a framework for understanding how energy is distributed and utilized in any isolated system․ The concept is applicable not only to heat engines but across all branches of physics and engineering, including electrical and mechanical systems․ Understanding this conservation principle is crucial to appreciate how energy is utilized, transferred, and transformed in various natural and technological processes․ This principle is a fundamental tool in understanding the physical world․
Heat, Work, and Internal Energy
Within the framework of thermodynamics, heat, work, and internal energy are crucial concepts that define the energy dynamics of a system․ Heat refers to the transfer of energy due to a temperature difference, typically from a hotter to a colder region․ Work, on the other hand, involves the transfer of energy when a force causes a displacement․ Internal energy represents the total energy contained within a system, encompassing kinetic and potential energies of its molecules․ The first law of thermodynamics establishes a direct relationship between these concepts, stating that the change in a system’s internal energy is equal to the heat added to the system minus the work done by the system․ This relationship offers a means to quantify energy exchanges within the system, allowing us to analyze diverse processes, from heating a substance to the expansion of a gas․ The interplay between heat, work, and internal energy is foundational to understanding the workings of heat engines, refrigerators, and other thermodynamic systems․ This relationship is fundamental to analyze and predict the behavior of energy in real-world applications․
The Second Law of Thermodynamics
The second law introduces the concept of entropy, governing the direction of natural processes․ It dictates that in an isolated system, entropy tends to increase over time, leading to a state of greater disorder or randomness․
The Concept of Entropy
Entropy, a central concept in the second law of thermodynamics, is often described as a measure of disorder or randomness within a system․ It quantifies the number of possible microscopic arrangements or microstates that correspond to a given macroscopic state․ A higher entropy value indicates a greater number of available microstates and, consequently, a higher degree of disorder․ Unlike energy, which is conserved according to the first law, entropy tends to increase in an isolated system over time, reflecting a natural tendency towards more probable and less ordered states․ This increase is not absolute, but rather a statistical trend, making it a crucial element in understanding irreversible processes․ Entropy’s role extends from physical systems to chemical reactions, providing insights into the direction and spontaneity of these processes, as they naturally move towards states of maximum entropy;
Entropy and System Disorder
The connection between entropy and system disorder is fundamental to understanding the second law of thermodynamics․ Entropy is not just an abstract concept but is directly related to how dispersed energy and matter are within a system․ A highly ordered system, such as a crystal, has low entropy because there are few ways to arrange its constituent particles while maintaining that order․ Conversely, a disordered system, like a gas expanding into a vacuum, has high entropy because there are countless ways to arrange its particles․ This relationship explains why systems naturally tend to evolve towards states of greater disorder, as these are statistically more probable․ The increase in entropy is synonymous with the spreading of energy, becoming more uniformly distributed among available microstates․ This tendency highlights the inherent directionality in thermodynamic processes, influencing everything from heat transfer to the unfolding of chemical reactions․
The Third Law of Thermodynamics
The third law addresses the behavior of entropy as a system approaches absolute zero․ It states that the entropy of a perfect crystal at absolute zero is exactly zero, providing a baseline for entropy calculations and highlighting the unattainability of absolute zero․
Absolute Zero and Entropy
The third law of thermodynamics introduces the concept of absolute zero, a theoretical temperature at which all molecular motion ceases, and provides a crucial understanding of entropy at this limit․ Specifically, the law asserts that the entropy of a perfectly crystalline substance approaches zero as the temperature approaches absolute zero (0 Kelvin or -273․15 degrees Celsius)․ This is because at absolute zero, there’s only one possible microstate for the system, representing perfect order․ The third law establishes a baseline for entropy, which allows for the determination of absolute entropy values for substances at any temperature․ It’s important to note that achieving absolute zero is practically impossible, but the third law is invaluable for calculating thermodynamic properties and understanding the behavior of matter at very low temperatures․ This law highlights the connection between temperature, molecular motion, and the degree of disorder (entropy) in a system and is essential in various fields such as low-temperature physics and materials science․
Limitations and Applications
While the laws of thermodynamics are incredibly powerful, they do have limitations; They apply primarily to macroscopic systems in equilibrium or undergoing reversible processes․ The laws don’t directly address microscopic phenomena or systems far from equilibrium, where statistical mechanics and other specialized approaches become necessary․ Furthermore, the laws are based on observations and do not provide a mechanism for the observed behaviors․ Despite these limitations, the applications of thermodynamics are vast and span across numerous fields․ They are fundamental in engineering for designing engines, refrigerators, and power plants․ In chemistry, they govern chemical reactions, phase transitions, and the stability of compounds․ Moreover, thermodynamics plays a critical role in understanding biological processes, materials science, and even cosmology․ By providing a framework for understanding energy and entropy, the laws of thermodynamics enable us to solve complex problems and advance technology in various sectors․ The laws are truly indispensable for understanding the world around us․